As people in many countries are rolling up their sleeves to get their COVID ‘shots’, there is much reason to celebrate medical science for developing a treatment in the shortest span in recorded history. Indeed, we have come a long way since Edward Jenner, the popularizer of the formal practice of vaccination, first administered a vaccine by collecting fluid from a cowpox-afflicted lady and injecting it directly into a boy. He then tested if he developed smallpox by inoculating him with the virus. Sacrilegious as it sounds, the fact is, the practice of medicine was not always as tightly regulated as it is now (for good reasons!) and indeed living a long, healthy life was only for the lucky few.
In most countries, if not all, a child will get vaccinated against at least 14 deadly diseases before the age of 10. Our world would almost seem like a panacea to our great grandparents who invariably saw their friends and family get afflicted and wiped out by what we now call preventable or curable diseases. Even so, the sad fact is many people still do suffer and die of preventable diseases due to inequality in access to medicine across the world (e.g. river blindness in Africa). Whereas most of the incurable diseases today seem to arise from genetic mishaps. Though COVID-19 formally still falls in the category of incurable diseases, given numerous educational resources available, it will be excluded from this discussion.
What makes a genetic disease hard to cure? In a genetic disease, a foreign pathogen does not simply attack an otherwise perfectly well-oiled machine, but something about DNA in our own cells makes us uniquely susceptible to certain infections (e.g. immunodeficiency disorders), or our own cells become the cause of plight (e.g. cancer, amyotrophic lateral sclerosis (ALS), Alzheimer’s). All of our cells contain the same DNA, and this DNA contains 4 different types of bases (A, C, G T) that are connected in a string-like fashion to create the code of life, called the DNA sequence or genome. Ever since you were a tiny, fused cell – your genome, half of which comes from a biological male, and the other half from a biological female, determined many of your traits. We ‘see’ only a few of the genetic traits manifest in how you look and behave because most genes are busy at work behind the curtains and keep you healthy and functioning the best they can. The human genome, or the entirety of the DNA sequence in an individual, contains ~6,200,000,000 bases. Strikingly, all humans presently on Earth share 99.9% DNA sequence. This fact is thrown around a lot, but it points to a crucial insight into evolution – that evolutionary mechanisms of checks and balances are so stringent, that only those of our ancestors who had near-perfect DNA sequences survived and passed on that near-perfect DNA to us. With this as the precedent, it would be even more shocking that sometimes our DNA is the source of ‘dis-ease’. How is that possible? How could we have ended up with such a faithful DNA optimization process and yet some mutations show up and survive despite their harmful impact on our health?
Mutations in DNA can arise spontaneously because of: errors in DNA replication or some parts of our DNA being prone to shifting their location (called ‘jumping’ or transposable elements) or environmental factors (certain chemicals or radiations can interact with our DNA). When these mutations occur in gametes, they are called germline mutations. Germline mutations stick and travel on to further generations, especially if they provide a survival advantage. If mutations occur in cells other than gametes, they cannot be passed onto the progeny and are called somatic mutations. It may seem counterintuitive to evolutionary design, but somatic mutations are a part of it too. To be ‘adaptive’ and optimal in responding to alterations in the environment, evolution selected for a way of changing DNA sooner than the reproduction cycle of an organism.

Both germline or somatic mutations may result in changes to the structure and function of proteins directly or indirectly. Some changes are beneficial, others could be harmful, and some are neutral to the organism.
Figure. 1. A single mutation C to T is shown in the DNA.
Source: https://www.sciencebuddies.org/teacher-resources/lesson-plans/genetic_mutation_PTC_test
Harmful mutations can wreak havoc in many ways: Firstly, by making cells divide uncontrollably, resulting in cancer; secondly, by making abnormal proteins that escape timely degradation and accumulate, as in the brain in Alzheimer’s disease thirdly, by making cells defective in working well on their particular assigned job, e.g. red blood cells delivering oxygen or B or T cells’ ability to fight germs.
An example of germline mutation is the Hbs mutation in a hemoglobin-producing gene that provides a survival advantage to tropical populations by making them resistant to malaria. A single copy of this mutation, i.e., either from mother or father is enough to provide this survival advantage. On the other hand, if one receives this mutation from both parents, this results in sickle cell disease (SCD) where the red blood cells develop an abnormal sickle-like shape, cannot carry oxygen effectively, and if pushed to the edge, results in blocked blood vessels (Figure 2, 3). Thus, a chance of survival advantage is inherently tied to a chance of disadvantage. Such evolutionary trade-offs are commonplace and theoretically provide a cumulative advantage to the species at large. Another important point to note here is that while all cells carry the germline mutation, that particular part of DNA might not necessarily play a major functional role in all cells. E.g. Hbs mutation does not change skin cell function or brain cell function in a major way. Hemoglobin is exclusively produced in red blood cells; therefore, the mutation creates abnormal protein only in red blood cells. This kind of tissue specificity has been useful in treating genetic disorders as we will discuss in brief later.
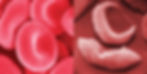
Figure. 2. Normal red blood cells vs. sickle red blood cells.
Source: https://www.britannica.com/science/sickle-cell-anemia

Figure. 3. Adapted from https://www.institutobernabeu.com/foro/en/can-avoid-passing-hereditary-diseases-children-taking-gct-genetic-compatibility-test/
How do we know whether our DNA may contain a harmful mutation? In principle, we have the wherewithal to identify diseases caused by a single mutation in your entire DNA. However, sequencing an entire genome is still very expensive and painstaking. So far, whole-genome sequencing has only been completed for 69 individuals. Therefore, the most well-characterized genetic disorders are tested using a panel with a possible set of mutations based on previous records of individuals with similar genetic disorders. This allows for sequencing a very small portion of the genome making it cost-effective and rapid for diagnostics.
Treatments for genetic disorders, even at a time when their origin was unknown, included removal of tissue of concern (e.g. in breast cancer), minimizing numbers of cells with mutations by blocking their replication, or targeting a part or whole of the tissue with specific drugs that will cause them to die and replacing with a healthy tissue if essential (e.g. in bone marrow transplant). However, each of these treatments has its own challenges. Removing tissues by surgery or drug/chemotherapeutic treatment only minimizes the severity or risk, but does not eliminate the root cause of disease – the mutation itself, and/or comes with additional risk and/or side effects of its own. Cells with mutations can still lurk around and diseases can recur – a common phenomenon in cancers.
A new form of therapy, called gene therapy, has shown promise in the last decade with intensive research efforts and clinical trials in place. The foundational principle of gene therapy is that sick cells can be made healthy by inactivating the defective gene, and if essential, adding a healthy gene - either the exact same gene or one that can carry out the same function (Figure 3). In this way, a genetic defect can be fixed at its source. Even though it sounds perfect, since the technique was first shown to work in cells in a dish in 1989, the road for gene therapy has been a twisted one. It has resulted in patient deaths due to high gene dosage that can trigger a hyperactive immune response and is still under acute scrutiny. Simultaneously, gene therapy trials for treating some diseases have been successful. While major challenges still remain, I will discuss why the future of gene therapy looks bright and what challenges still remain, using two recent clinical trials as examples.
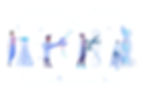
Figure 3. Scientists can now synthesize, edit, delete and insert new parts of DNA into the genome. Source: https://answers.childrenshospital.org/gene-therapy-history/
In both the trials discussed below, gene therapy was designed for patients with SCD who have a defective form of adult hemoglobin. So, let’s talk a little bit about how hemoglobin is formed. In early developmental stages, that is, in embryos and early childhood, a gene called fetal globin makes most of the hemoglobin required for oxygen delivery to all the tissues. At this stage, the other type of hemoglobin, called adult hemoglobin, is switched off. Then a gene called BCL11A, which plays the role of ‘switch’, turns off the gene that makes fetal hemoglobin and turns on the adult hemoglobin. Consequently, the fetal hemoglobin remains inactive for the rest of adult life. The mutation that causes sickle cell disease occurs in the adult hemoglobin gene. In young children, sickle cell disease (SCD) begins to manifest in the form of bouts of pain due to blood vessel blocks, and difficulty breathing or sustained physical activity – a common consequence of ineffective oxygen delivery by red blood cells. Given that the fetal hemoglobin functions perfectly in these individuals, scientists came up with the brilliant idea of turning the fetal counterparts back on and turning adult hemoglobin off by getting rid of the ‘switch’ BCL11A gene. Scientists have spent decades in formulating and optimizing this ingenious approach. It is important to emphasize that basic science research is an integral component of therapy development. Both the therapy trials discussed below employed 'turning off the BCL11A switch to turn fetal hemoglobin on' as the end goal albeit using different means to achieve it.
In a phase one trial conducted by bluebird bio in collaboration with Boston Children’s Hospital, scientists first isolated stem cells from the patient and modified them to compensate for the defective gene. To add the gene, they used a carrier called ‘lentiviral vector’ which has a virus-like backbone DNA in it, but instead of harmful viral genes, it contains a sequence of interest to the scientist. Lentiviral backbones are special in that they can integrate their DNA into the host DNA. In a virus, it provides the advantage of easily having viral genes be expressed inside the host that in turn make more viruses. Lentiviral vectors benefit from this advantage, but for making more copies of the DNA of interest. Here, the DNA sequence of interest is specifically designed to turn the switch gene BCL11A off. This would lead to turning on of the fetal hemoglobin gene that works perfectly in these patients and could restore normal red blood cell function. Then the doctors rid the patient of sick cells by irradiating the bone marrow. Bone marrow, the site of origin for all blood cells, is extremely sensitive to irradiation so that nearly all blood-forming stem cells are killed. Then the ‘corrected’ stem cells were transplanted back into the patients. This had a striking impact in restoring fetal globin in patients. As of Dec 2020, five of six patients that were followed up for more than six months showed normal hemoglobin levels. However, one patient continued to be transfusion-dependent after therapy. In all cases, little to no events of ‘pain crises’ were reported, and the general health of the patients improved.
A major challenge for viral vectors has been that the integration into the human genome can non-specifically activate or inactivate other genes which in turn can be detrimental (e.g. activation of cancer-causing genes). Although lentiviral vectors have a better chance over other types of viral vectors in this regard, the risk is not zero. Prior use of lentiviral vectors for SCD and other diseases has produced variable results, thus, confidence in this approach still remains to be solidified. In Feb 2021, bluebird bio reported that two patients enrolled in a different trial using the same vector, but a different way to treat SCD, presented with blood-related problems. The company responded by halting the trial and conducting the further assessment. Analyses revealed that the lentiviral vector may not be the cause for at least one of the two cases.
In another trial by CRISPR Therapeutics and Vertex Pharmaceuticals, a genome-editing approach called CRISPR, invented less than a decade ago, was used instead of a viral vector to silence the switch gene BCL11A. This was the first time using CRISPR in any documented trial. Blood-forming cells were first obtained from healthy donors, and a sequence responsible for BCL11A function (but not a part of its gene sequence) was deleted using CRISPR-Cas9. Strikingly, the effectiveness of this strategy in deleting the sequence was up to 80%. Then these modified cells were transplanted into three SCD patients. In a report published in Dec 2020 and a plenary session at the ASH 2020 conference, all the patients exhibited a stable increase in fetal hemoglobin and reported elimination of the painful episodes more than a year after their respective transplants.
Encouraged by these data, on March 30th, 2021, the US FDA (Food and Drug Administration) approved the start of another CRISPR-based clinical trial for SCD, recruitment for which will start in summer 2021. This trial will be conducted under the collaborative efforts of the Innovative Genomics Institute (IGI) and the University of California Los Angeles (UCLA). Dr. Jennifer Doudna, one of the co-inventors of CRISPR-Cas9 technology and the founder of IGI, will co-lead the trial. In this trial, scientists plan to use the patient’s own cells for editing and transplantation instead of healthy donors.
Looking forward, biomedical research and advancement have made it possible to treat diseases like HIV in a way previously unthinkable. In addition to treating SCD, the most prevalent genetic disease in humans, intensive efforts are underway to develop gene therapy to treat rare but deadly childhood diseases. Further improvement of gene therapy will revolutionize medicine and potentially could treat a large host of ‘incurable’ diseases.
References: